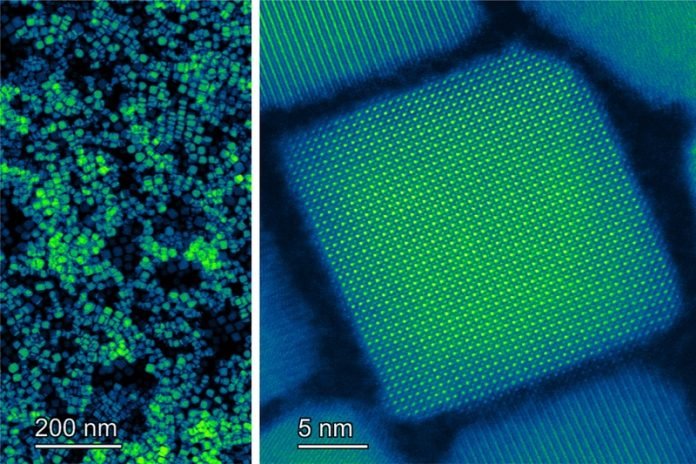
Using novel materials that have been widely studied as potential new solar photovoltaics, researchers at MIT have shown that nanoparticles of these materials can emit a stream of single, identical photons.
While the work is currently a fundamental discovery of these materials’ capabilities, it might ultimately pave the way to new optically based quantum computers, as well as possible quantum teleportation devices for communication, the researchers say.
The results appear in the journal Nature Photonics, in a paper by graduate student Alexander Kaplan, professor of chemistry Moungi Bawendi, and six others at MIT.
Most concepts for quantum computing use ultracold atoms or the spins of individual electrons to act as the quantum bits, or qubits, that form the basis of such devices.
But about two decades ago some researchers proposed the idea of using light instead of physical objects as the basic qubit units.
Among other advantages, this would eliminate the need for complex and expensive equipment to control the qubits and enter and extract data from them. Instead, ordinary mirrors and optical detectors would be all that was needed.
“With these qubit-like photons,” Kaplan explains, “with just ‘household’ linear optics, you can build a quantum computer, provided you have appropriately prepared photons.”
The preparation of those photons is the key thing. Each photon has to precisely match the quantum characteristics of the one before, and so on.
Once that perfect matching is achieved, “the really big paradigm shift then is changing from the need for very fancy optics, very fancy equipment, to needing just simple equipment. The thing that needs to be special is the light itself.”
Then, Bawendi explains, they take these single photons that are identical and indistinguishable from each other, and they interact them with each other. That indistinguishability is crucial: If you have two photons, and “everything is the same about them, and you can’t say number one and number two, you can’t keep track of them that way. That’s what allows them to interact in certain ways that are nonclassical.”
Kaplan says that “if we want the photon to have this very specific property, of being very well-defined in energy, polarization, spatial mode, time, all of the things that we can encode quantum mechanically, we need the source to be very well-defined quantum mechanically as well.”
The source they ended up using is a form of lead-halite perovskite nanoparticles. Thin films of lead-halide perovskites are being widely pursued as potential next-generation photovoltaics, among other things, because they could be much more lightweight and easier to process than today’s standard silicon-based photovoltaics.
In nanoparticle form, lead-halide perovskites are notable for their blindingly fast cryogenic radiative rate, which sets them apart from other colloidal semiconductor nanoparticles.
The faster the light is emitted, the more likely the output will have a well-defined wavefunction. The fast radiative rates thus uniquely position lead-halide perovskite nanoparticles to emit quantum light.
To test that the photons they generate really do have this indistinguishable property, a standard test is to detect a specific kind of interference between two photons, known as Hong-Ou-Mandel interference.
This phenomenon is central to a lot of quantum-based technologies, Kaplan says, and therefore demonstrating its presence “has been a hallmark for confirming that a photon source can be used for these purposes.”
Very few materials can emit light that meets this test, he says. “They pretty much can be listed on one hand.” While their new source is not yet perfect, producing the HOM interference only about half the time, the other sources have significant issues with achieving scalability.
“The reason other sources are coherent is they’re made with the purest materials, and they’re made individually one by one, atom by atom. So, there’s very poor scalability and very poor reproducibility,” Kaplan says.
By contrast, the perovskite nanoparticles are made in a solution and simply deposited on a substrate material. “We’re basically just spinning them onto a surface, in this case just a regular glass surface,” Kaplan says. “And we’re seeing them undergo this behavior that previously was seen only under the most stringent of preparation conditions.”
So, even though these materials may not yet be perfect, “They’re very scalable, we can make a lot of them. and they’re currently very unoptimized. We can integrate them into devices, and we can further improve them,” Kaplan says.
At this stage, he says, this work is “a very interesting fundamental discovery,” showing the capabilities of these materials. “The importance of the work is that hopefully it can encourage people to look into how to further enhance these in various device architectures.”
And, Bawendi adds, by integrating these emitters into reflective systems called optical cavities, as has already been done with the other sources, “we have full confidence that integrating them into an optical cavity will bring their properties up to the level of the competition.”
The research team included Chantalle Krajewska, Andrew Proppe, Weiwei Sun, Tara Sverko, David Berkinsky, and Hendrik Utzat. The work was supported by the U.S. Department of Energy and the Natural Sciences and Engineering Research Council of Canada.
Written by David L. Chandler.