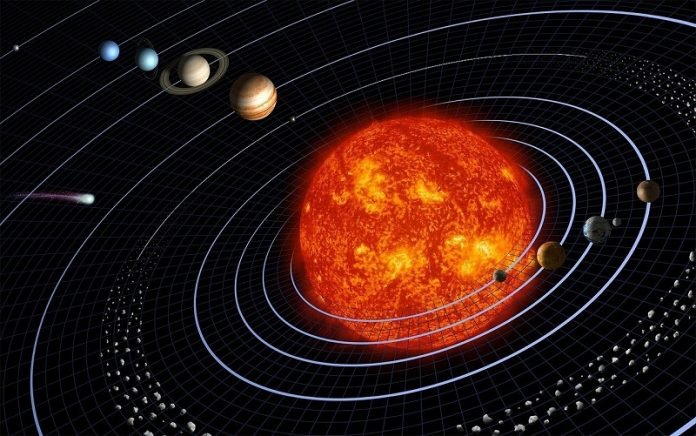
The motion of a tiny number of charged particles may solve a longstanding mystery about thin gas disks rotating around young stars, according to a new study from Caltech.
These features, called accretion disks, last tens of millions of years and are an early phase of solar system evolution.
They contain a small fraction of the mass of the star around which they swirl; imagine a Saturn-like ring as big as the solar system.
They are called accretion disks because the gas in these disks spirals slowly inward toward the star.
Scientists realized long ago that when this inward spiraling occurs, it should cause the radially inner part of the disk to spin faster, according to the law of the conservation of angular momentum.
To understand conservation of angular momentum, think of spinning figure skaters: when their arms are outstretched, they spin slowly, but as they draw their arms in, they spin faster.
Angular momentum is proportional to velocity times radius, and the law of angular momentum conservation states that the angular momentum in a system stays constant.
So, if the skater’s radius decreases because they have drawn their arms in, then the only way to keep angular momentum constant is to increase the spin velocity.
The inward spiral motion of the accretion disk is akin to a skater drawing their arms in—and as such, the inner part of the accretion disk should spin faster.
Indeed, astronomical observations show that the inner part of an accretion disk does spin faster. Curiously, though, it does not spin as fast as predicted by the law of conservation of angular momentum.
Over the years, researchers have investigated many possible explanations for why accretion disk angular momentum is not conserved. Some thought friction between the inner and outer rotating parts of the accretion disk might slow down the inner region.
However, calculations show that accretion disks have negligible internal friction.
The leading current theory is that magnetic fields create what is called a “magnetorotational instability” that generates gas and magnetic turbulence—effectively forming friction that slows down the rotational speed of inward spiraling gas.
“That concerned me,” says Paul Bellan, professor of applied physics. “People always want to blame turbulence for phenomena they do not understand. There’s a big cottage industry right now arguing that turbulence accounts for getting rid of angular momentum in accretion disks.”
A decade and a half ago, Bellan began investigating the question by analyzing the trajectories of individual atoms, electrons, and ions in the gas that constitutes an accretion disk.
His goal was to determine how the individual particles in the gas behave when they collide with each other, as well as how they move in between collisions, to see if angular momentum loss could be explained without invoking turbulence.
As he explained over the years in a series of papers and lectures that were focused on “first principles”—the fundamental behavior of the constituent parts of accretion disks—charged particles (i.e., electrons and ions) are affected by both gravity and magnetic fields, whereas neutral atoms are only affected by gravity. This difference, he suspected, was key.
Caltech graduate student Yang Zhang attended one of those talks after taking a course in which he learned how to create simulations of molecules as they collide with each other to produce the random distribution of velocities in ordinary gases, such as the air we breathe.
“I approached Paul after the talk, we discussed it, and ultimately decided that the simulations might be extended to charged particles colliding with neutral particles in magnetic and gravitational fields,” Zhang says.
Ultimately, Bellan and Zhang created a computer model of a spinning, super-thin, virtual accretion disk. The simulated disk contained around 40,000 neutral and about 1,000 charged particles that could collide with each other, and the model also factored in the effects of both gravity and a magnetic field.
“This model had just the right amount of detail to capture all of the essential features,” Bellan says, “because it was large enough to behave just like trillions upon trillions of colliding neutral particles, electrons, and ions orbiting a star in a magnetic field.”
The computer simulation showed collisions between neutral atoms and a much smaller number of charged particles would cause positively charged ions, or cations, to spiral inward toward the center of the disk, while negatively charged particles (electrons) spiral outward toward the edge. Neutral particles, meanwhile, lose angular momentum and, like the positively charged ions, spiral inward to the center.
A careful analysis of the underlying physics at the subatomic level—in particular, the interaction between charged particles and magnetic fields—shows that angular momentum is not conserved in the classical sense, though something called “canonical angular momentum” is indeed conserved.
Canonical angular momentum is the sum of original ordinary angular momentum plus an additional quantity that depends on the charge on a particle and the magnetic field. For neutral particles, there is no difference between ordinary angular momentum and canonical angular momentum, so worrying about canonical angular momentum is unnecessarily complicated.
But for charged particles—cations and electrons—the canonical angular momentum is very different from the ordinary angular momentum because the additional magnetic quantity is very large.
Because electrons are negative and cations are positive, the inward motion of ions and outward motion of electrons, which are caused by collisions, increases the canonical angular momentum of both. Neutral particles lose angular momentum as a result of collisions with the charged particles and move inward, which balances out the increase in the charged-particle canonical angular momentum.
It is a small distinction, but makes a huge difference on a solar system-wide scale, says Bellan, who argues that this subtle accounting satisfies the law of conservation of canonical angular momentum for the sum of all particles in the entire disk; only about one in a billion particles needs to be charged to explain the observed loss of angular momentum of the neutral particles.
Furthermore, Bellan says, the inward motion of cations and outward motion of electrons results in the disk becoming something like a gigantic battery with a positive terminal near the disk center and a negative terminal at the disk edge.
Such a battery would drive electric currents that flow away from the disk both above and below the plane of the disk. These currents would power astrophysical jets that shoot out from the disk in both directions along the disk axis.
Indeed, jets have been observed by astronomers for over a century and are known to be associated with accretion disks, though the force behind them has long been a mystery.
Bellan and Yang’s paper is titled “Neutral-charged-particle Collisions as the Mechanism for Accretion Disk Angular Momentum Transport,” and was published in the Astrophysical Journal.